Exploring the Structural Biology of Evolution
Evolution is a natural process that produces improved organisms without any need for intelligent intervention.
Evolution is a natural process where organisms compete in whatever environmental niche they occupy, and the best ones survive and succeed in the "game of life.” Organisms that are less able to compete go extinct. All of this happens without the need for intelligent intervention or design, albeit very slowly most of the time. Scientists believe that all species of living organisms on Earth arose through evolution from simple primordial unicellular organisms. Looking at the structures of biological molecules, we can explore how evolution has shaped modern proteins and nucleic acids, and search for clues about the molecular nature of the first living things. Scientists are also mimicking the natural processes of evolution in the laboratory, using it to improve the functional properties of proteins and nucleic acids under “artificial” selection pressure.
Access the individual sections in this resource
1. Variation and Selection
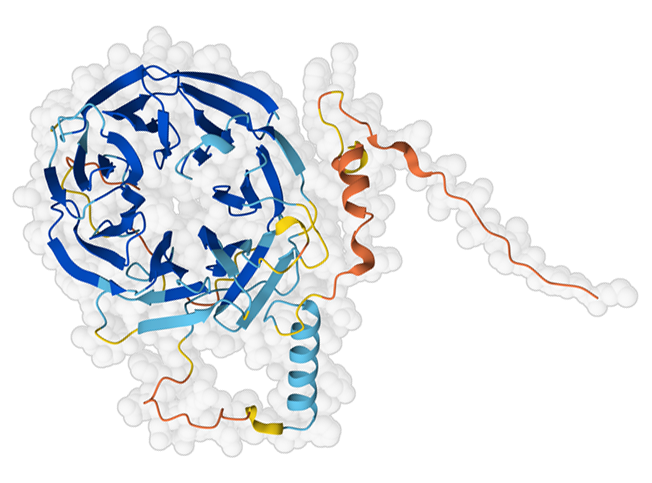
Computed structure model of cortex protein, which controls the coloration of wings in the peppered moth. Well-predicted portions are in blue and poorly-predicted portions are in orange. PDB ID AF_AFP0DOC0F1.
The process of evolution requires two things: a population of organisms undergoing random genetic variation due errors made during DNA replication and other processes, and a selection pressure that retains the most successful variants and removes the others.
The peppered moth is a classic example of evolution that was observed in action on a very short time scale (compared to more typical evolutionary processes). A protein called cortex controls the color of these moths. They’re normally bright white with black peppering, and are difficult to see on typical tree bark.
But during the industrial revolution, trees became covered with black soot, and these bright moths became easy prey for birds despite the peppering. However, this moth population included rare variants that are completely black, caused by differences in the gene encoding the cortex protein that leads to overproduction of the protein. The dark moths, with the unusual cortex gene, soon dominated the population since they were less visible to birds. In this way, the population evolved from white moths to black moths in response to a change in environmental conditions.
2. Reconstructing the Tree of Life
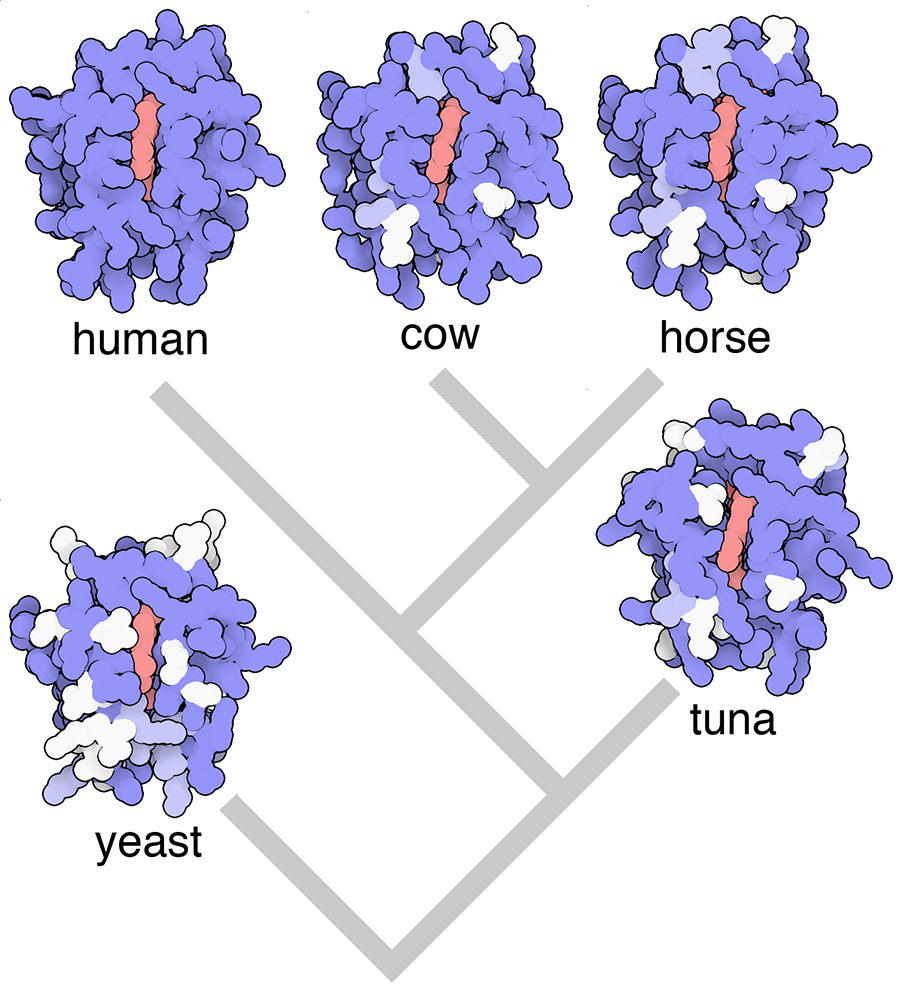
Evolution of cytochrome c. Variations in the protein from different organisms are compared to the human form, with small, conservative changes in light blue and larger changes in white. By counting up the number of changes, we can see that yeast is more distantly related to us than the other three animals. PDB IDs 1cyc, 1hrc, 1ycc, 2b4z, 3zcf.
We can reconstruct the timeline of evolution by looking for similarities between organisms, and in even more detail, by looking at similarities between proteins. For example, we can build a tree of life by noting that horses and cows are very similar, with four legs, and tuna are different, having fins. So, we infer that all three evolved from a primordial animal early in evolution, but the horses and cows diverged later from a common horse-like ancestor.
By looking at proteins from these animals, we can get a more accurate timeline. Typically, scientists use essential proteins like cytochrome c to study these relationships, so that the slow accumulation of genetic changes leading to amino acid changes in the protein are easier to quantify.
3. Gene Duplication
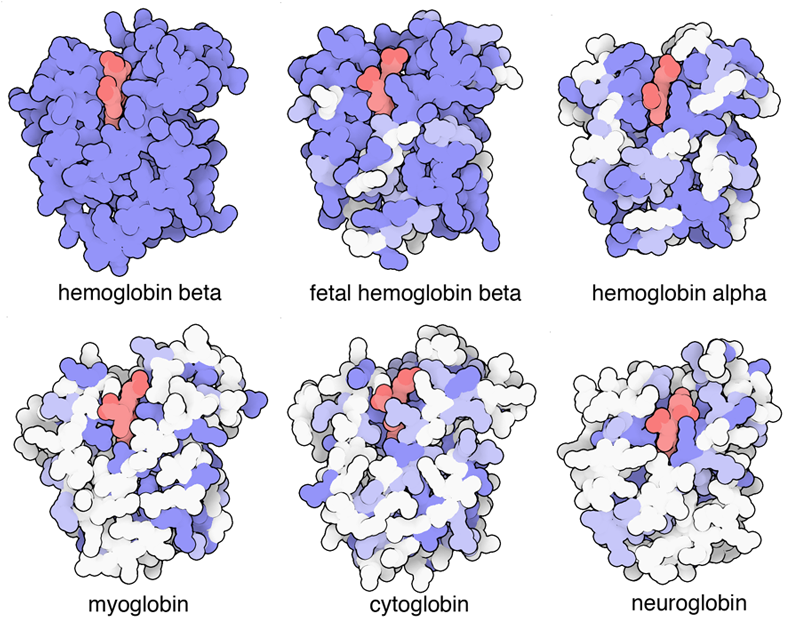
Human globins encoded in the human genome are thought to have evolved by gene duplication from a common ancestral protein. Similarities in protein sequence are colored as in the previous figure, with hemoglobin beta as the reference. Notice that the amino acids around the heme (red) are the most conserved portions of the proteins. PDB IDs 1fdh, 1hho, 1oj6, 1ut0, 3rgk.
Evolution typically proceeds by selecting for individuals in populations of organisms with mutated proteins that confer a competitive advantage. This can be tricky, however, since each cell needs most of its proteins to survive, and most changes cause big problems. Because of this, proteins often evolve by duplicating the genes that encode the protein. The two copies of the gene can then diverge, one continuing to perform its normal function and the other free to change and adopt a new function.
Many examples of this type of duplication and divergence are found in our cells. For example, our cells build many types of globins, all of which are thought to have arisen by duplication from a single ancestral gene. Other familiar examples are the digestive enzymes trypsin, chymotrypsin, and elastase, which all have similar structures but cleave slightly different types of proteins.
4. Convergent Evolution
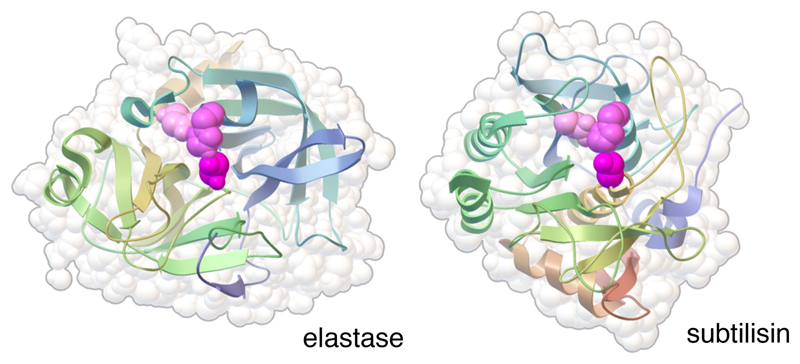
Convergent evolution of serine proteases. The active site triad is shown in magenta, with serine in the brightest color. Note that the triad is structurally similar, but the overall folds of the two proteins are entirely different. PDB IDs 3est, 1scn.
The random process of evolution occasionally stumbles upon different solutions to the same problem. For example, our eyes and octopus eyes both detect light, but they are thought to have evolved independently. Looking at proteins, we can find examples of convergent evolution.
For example, the characteristic active site catalytic triad of serine proteases has evolved independently multiple times. Antifreeze proteins, which bind to the surface of ice crystals, are another example of convergent evolution.
5. Evolution in Action
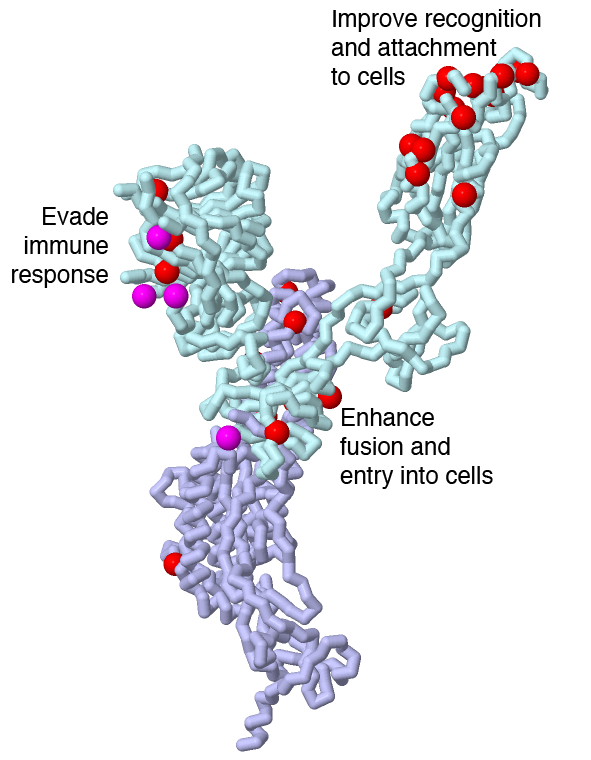
Mutations in an omicron variant of SARS-CoV-2 spike. Mutation sites are in red and deletion sites are in magenta. Changes in different regions are thought to provide different functional advantages for the virus. PDB ID 7t9k.
The evolution of organisms such as ourselves occurs over vast time periods, through slow accumulation of selected genetic variations. However, evolution also occurs at very rapid rates in cases where variation, reproduction, and the process of natural selection occurs more quickly.
The entire world witnessed this phenomenon during the COVID-19 pandemic. The spike protein rapidly mutated within the huge global population of viruses, and the most infectious variants were repeatedly selected and generated waves of successive infection. Structures of these variant spike proteins pinpoint the sites that mutate, which improve the function of the spike in multiple ways.
6. Evolution in the Laboratory
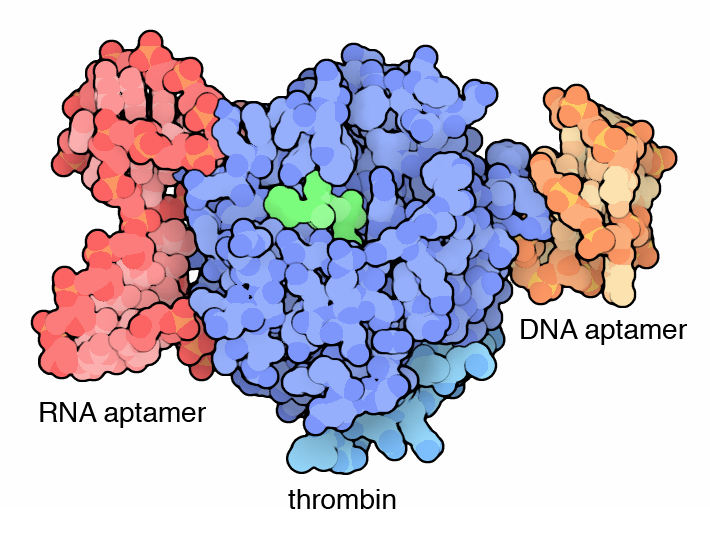
Artificial aptamers that bind to the enzyme thrombin were discovered by artificial evolution of many variant forms of the molecules. PDB IDs 1hut, 4i7y.
The idea of biological evolution is being applied in the laboratory to discover molecules with desired properties. The process is similar to natural evolution. Many, many random variants are created and the best ones are selected. Then these best ones are randomly modified, and the best are selected once again. After repeating this process, highly functional molecules are found.
Turnkey laboratory methods for this type of artificial variation and selection are available for RNA and DNA, creating “aptamers” with defined functions. The same process is also being applied to enzymes, starting from an existing natural enzyme and then performing many rounds of laboratory evolution to identify a new enzyme with a different function but a similar 3D structure.
7. RNA and the Origin of Life
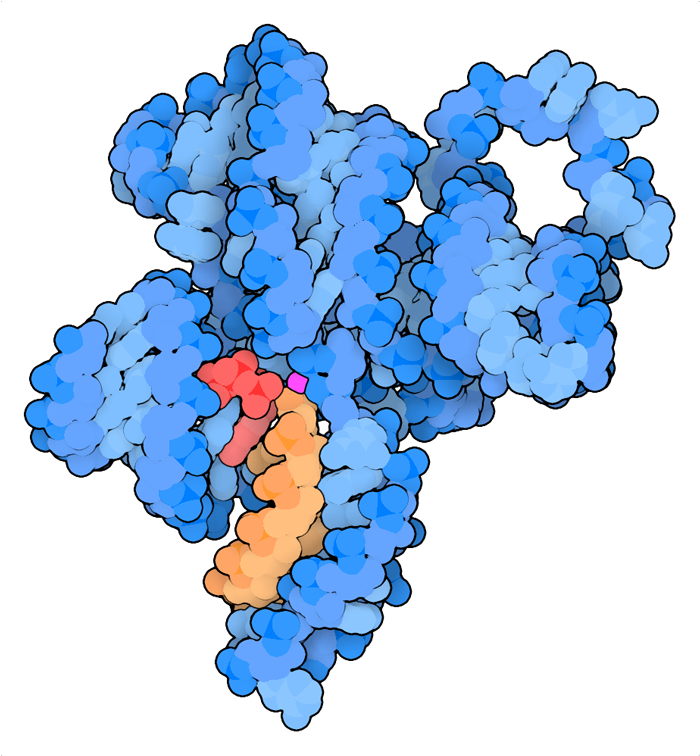
RNA ligase ribozyme. This structure includes an artificial ribozyme (blue) that can connect a nucleotide (red) to an RNA chain (orange) with the help of a magnesium ion (magenta). PDB ID 3r1h.
By comparing biomolecules inside many types of cells, researchers are trying to reconstruct the nature of the very first living organisms on Earth. One particularly compelling observation is that much of the basic cellular machinery for protein synthesis is composed of RNA. Ribosomes catalyze their protein-building reactions using RNA nucleotides, not protein, and transfer RNA is the key to translation, linking the code of nucleotides to the code of amino acids.
For this reason, many researchers think that life started in a world of self-replicating RNA. To explore this idea, researchers are creating RNA molecules that act like this early RNA-based life, such as ribozymes that act as RNA ligases and polymerases.
For more information, see:
-
Bordin, N., Sillitoe, I., Lees, J.G., Orengo, C. (2021) Tracing evolution through protein structure: Nature captured in a few thousand folds. Frontiers in Molecular Biosciences 8, 668184.
-
van’t Hof, A.E., Campagne, P., Rigden, D.J., Yung, C.J., Lingley, J., Quail, M.A., Hall, N., Darby, A.C., Saccheri, I.J. (2016) The industrial melanism mutation in British peppered moths is a transposable element. Nature 534, 102-105.
-
Higgs, P.G., Lehman, N. (2014) The RNA World: Molecular cooperation at the origins of life. Nature Reviews Genetics 16, 7-17.